
Case study1-Please Use your keyboard (Don’t use handwriting)Thank you.2-I need new an unique answers,please(Use your own words,don’t copy and past)3-The answer must be over 600 words4-Please provide more than 5 reference with apa reference,and if you can one of those reference should be from the book called (Management: A Practical Approach” 9th edition by Kinicki, A., & Williams, B.), please so i can get full mark .5-No plagiarism6-All answered must be typed using Times New Roman (size 12, double-spaced) font7-Please read the case “New York’s Subway System Is Crumbling” on the pdf file and answer the questions on the (word) file that i gave them to youBiology 108 Discussion #5 Antimicrobial & Insecticide Resistance Read: (1) Antibiotic use in 2016 – from Center for Diseases Control (CDC) report: Antibiotic Use in the United States, 2018 Update: Progress and Opportunities (1 page). (2) Christaki et al (2020 J. Mol. Evolution) – READ ONLY: pp26-28 top (Abstract to end of Antimicrobial Resistance: Intrinsic, Acquired, and Adaptive) AND pp33-35 (Start of Evolution of Antimicrobial Resistance to end of Fitness Restoring Compensatory Mutations). LOOK AT the list of Antibiotic Resistance Mechanisms (left column, Table 1). (3) Silbergeld et al (2008 Ann. Rev. Public Health) – Abstract & Introduction. (3 pages) (4) Heckel (2012 Science) (3 pages) (5) Georghiou & Taylor (1986) (5 pages) (6) Tabashnik (2008 PNAS) (2 pages) (7) Georghiou (1986) Table Questions to answer Antibiotic Resistance. 1. Why should we care about microbial resistance to antibiotics (more generally called antimicrobials)? What factors are driving the development of resistance? (Articles #1, #2 & #3). 2. What are the origins of antimicrobial resistance and how does it spread? (Article #2) 3. The use of antimicrobials in farm animals for non-therapeutic purposes (such as improving growth) was banned in Europe in 2006 and in the US in 2017. Why was this considered important? (Article #3). Insecticide Resistance. 4. Who was Rachel Carson? She recognized that the use of insecticides makes the problem worse over the long term – explain. (Article #4) 5. Insecticide resistance is widespread, and in pest insects (which are typically diploid) resistant/susceptible heterozygotes are usually of intermediate resistance. This leads to the possibility of “functional dominance”. What is this? It is important for an important strategy for slowing down the spread of resistance, the high dose refuge strategy. What is the high dose refuge strategy? (Articles #5 & #6) 6. What is Bt and what are Bt crops? (Articles #4 & #6) Both 7. What is fitness compensation? It is seen in antibiotic resistant bacteria and insecticide resistant insects. (Articles #2, #5, #7). 3 Data for Action One of the most important ways CDC helps improve antibiotic use is producing and analyzing data to support healthcare facilities and providers in making the best choices for their patients. Healthcare facilities, providers, health departments, and other partners can use these data to identify opportunities to improve antibiotic stewardship efforts. 2016 Outpatient Antibiotic Prescribing Data Too many antibiotics are prescribed unnecessarily in the United States. CDC estimates about 47 million antibiotic courses each year are prescribed for infections that don’t need antibiotics in U.S. doctors’ offices and emergency departments each year. That’s about 30% of all antibiotics prescribed. Antibiotic prescribing nationally has improved, with a 5% decrease from 2011 to 2016, but more progress needs to be made. In 2016, 270.2 million antibiotic prescriptions were written in the United States. That’s enough antibiotic courses for five out of every six Americans (on average) to receive an antibiotic prescription. Prescribing rates vary by state, with a 2.5-fold difference between the lowest and prescribing states, suggesting there are opportunities for improvement. Community Antibiotic Prescriptions by State—2016 65–101 105–130 131–145 147–163 164–193 201–305 Prescriptions per 1,000 Prescriptions per 1,000 Prescriptions per 1,000 511–640 687–744 751–852 855–905 911–964 997–1270 Community Antibiotic Prescriptions by State 2016 Percentage 38–66 67–78 79–84 >84 Percentage of Hospitals Meeting all 7 core elements by state 2017 324–600 626–716 722–809 811–896 918–998 1007–1341 Antibiotic prescribing rates per 1000 by state, 2013, ages 0 to 19 Azithromycin prescribing rates per 1000 by state, 2013, ages 0 to 19 Vol:.(1234567890) Journal of Molecular Evolution (2020) 88:26–40 https://doi.org/10.1007/s00239-019-09914-3 1 3 REVIEW Antimicrobial Resistance in Bacteria: Mechanisms, Evolution, and Persistence Eirini Christaki1,3 · Markella Marcou1,2 · Andreas Tofarides3 Received: 3 May 2019 / Accepted: 2 October 2019 / Published online: 28 October 2019 © Springer Science+Business Media, LLC, part of Springer Nature 2019 Abstract In recent years, we have seen antimicrobial resistance rapidly emerge at a global scale and spread from one country to the other faster than previously thought. Superbugs and multidrug-resistant bacteria are endemic in many parts of the world. There is no question that the widespread use, overuse, and misuse of antimicrobials during the last 80 years have been associated with the explosion of antimicrobial resistance. On the other hand, the molecular pathways behind the emergence of antimicrobial resistance in bacteria were present since ancient times. Some of these mechanisms are the ancestors of current resistance determinants. Evidently, there are plenty of putative resistance genes in the environment, however, we cannot yet predict which ones would be able to be expressed as phenotypes in pathogenic bacteria and cause clinical disease. In addition, in the presence of inhibitory and sub-inhibitory concentrations of antibiotics in natural habitats, one could assume that novel resistance mechanisms will arise against antimicrobial compounds. This review presents an overview of antimicrobial resistance mechanisms, and describes how these have evolved and how they continue to emerge. As antimicrobial strategies able to bypass the development of resistance are urgently needed, a better understanding of the critical factors that contribute to the persistence and spread of antimicrobial resistance may yield innovative perspectives on the design of such new therapeutic targets. Keywords Antimicrobial resistance · Evolution · Genomics · Antibiotics · Persistence Introduction Antibiotic resistance occurs when bacteria have or develop the ability to circumvent the mechanisms, which drugs use against them. Infections caused by antibiotic-resistant pathogens are usually more difcult to treat, and can relapse and cause signifcant morbidity and mortality. Epidemiological surveillance networks in Europe and Asia [European Antimicrobial Resistance Surveillance Network -EARS-Net, Central Asia and Eastern European Surveillance of Antimicrobial Resistance-(CAESAR)] have documented that antibiotic-resistant bacteria have become much more prevalent during the last decade (European Centre for Disease Prevention and Control 2018; World Health Organization 2017). Also, according to the Centers for Disease Control and Prevention (CDC), each year in the US, at least 2 million people get infected with antibiotic-resistant bacteria, and at least 23,000 people die as a result of these infections (CDC 2013). It is estimated that globally approximately 700,000 deaths are attributed annually to antimicrobial resistance and this could rise to 10 million deaths per year by 2050 (O’Neill 2014). Additionally, infections due to antimicrobial-resistant bacteria like those caused by multidrug-resistant Acinetobacter baumannii, Klebsiella pneumoniae, and methicillinresistant Staphylococcus aureus (MRSA) result in longer duration of hospitalization and pose a signifcant economic burden on national healthcare systems. Antimicrobial resistance, however, is associated with the widespread use and misuse of antibiotics, in humans, agriculture, animal farming, and industry (Harbarth et al. 2015) and thus needs to be viewed under a One-Health approach, as human health is inextricably linked to the health of Handling Editor: Konstantinos Voskarides. * Eirini Christaki [email protected] 1 Medical School, University of Cyprus, Nicosia, Cyprus 2 Microbiology Department, Archbishop Makarios III Hospital, Nicosia, Cyprus 3 Department of Medicine, Nicosia General Hospital, Nicosia, Cyprus Journal of Molecular Evolution (2020) 88:26–40 27 1 3 animals and the viability of ecosystems. This article aims to highlight the most important mechanisms of antimicrobial resistance as well as describe how these have emerged and evolved and what drives their persistence over time. A better understanding of the evolutionary drivers of antimicrobial resistance may ofer novel perspectives on ways to tackle this critical public health threat. Antimicrobials and Antimicrobial Resistance: A Brief History Penicillin, discovered by Alexander Fleming in 1928, was the frst antibiotic used successfully to control bacterial infections in soldiers during World War II. However, even before the use of penicillin, in 1940, the frst penicillinresistant Staphylococcus strains had already been described. To counteract the frst penicillinases, methicillin was introduced in 1959 and one year later, in 1960, a methicillinresistant Staphylococcus strain was reported (Sengupta et al. 2013). Vancomycin was introduced in 1958 for the treatment of methicillin-resistant staphylococci. A couple of decades later, in 1979, coagulase-negative staphylococci resistant to vancomycin were reported and ten years later resistance in enterococci was described (Courvalin 2006), followed by, in 1997, the report of less-susceptible S. aureus (vancomycin-intermediate S. aureus, VISA) strains in Japan (Levine 2005). Another historical example is tetracycline, which was introduced in 1950 followed by tetracycline-resistant Shigella strains being reported in 1959. Furthermore, levofoxacin was introduced into clinical practice in 1996 and in the same year levofoxacin-resistant pneumococcus was reported (Sengupta et al. 2013). For two decades, between 1960 and 1980, there was a seemingly adequate production of new antimicrobials by the pharmaceutical industry. However, after the 1980s, the rate of discovery of new antibiotic classes had dramatically decreased, until recently, when a new interest has sparked (Parmar et al. 2018). As a consequence of increasing antimicrobial resistance and a thin new antimicrobial pipeline, bacterial infections due to multidrug-resistant or extensively drug-resistant pathogens have become a major concern in clinical practice worldwide. Antimicrobial Resistance: Intrinsic, Acquired, and Adaptive Antibiotic resistance exhibited by bacteria can be intrinsic, acquired, or adaptive (Joon-Hee 2019). Intrinsic resistance is defned as the resistance exhibited due to the inherent properties of the bacterium. Examples of intrinsic resistance include the glycopeptide resistance exhibited by Gram-negative bacteria due to the impermeability of the outer membrane present in the Gram-negative bacterial cell envelope. Acquired resistance is defned as the resistance exhibited when a previously sensitive bacterium acquires a resistance mechanism by either a mutation or the acquisition of new genetic material from an exogenous source (horizontal gene transfer). Horizontal gene transfer can occur through three main mechanisms (Holmes et al. 2016; Munita and Arias 2016). Transformation: This is a form of genetic recombination in which free DNA fragments from a dead bacterium enter a recipient bacterium and are incorporated into its chromosome. Only a few bacteria are naturally transformable. Transduction: Transduction involves the transfer of genetic material between a donor and a recipient bacterium by a bacteriophage. Conjugation: This is probably the most important mechanism of horizontal gene transfer. It involves the transfer of genetic material from one bacterial cell to another by direct physical contact between the cells. A sex pillus forms between the two bacterial cells through which a plasmid is transferred from the donor cell to the recipient cell. Multiple resistance genes are often present on a single plasmid enabling the transfer of multidrug resistance in a single conjugation event. The assembly of multiple resistance genes on a single plasmid is mediated by mobile genetic elements (transposons, integrons, and Insertion Sequence Common Region- ISCR-elements). Adaptive resistance is defned as the resistance to one or more antibiotics induced by a specifc environmental signal (e.g., stress, growth state, pH, concentrations of ions, nutrient conditions, sub-inhibitory levels of antibiotics). In contrast to intrinsic and acquired resistance, adaptive resistance is transient. Adaptive resistance, which allows bacteria to respond more rapidly to antibiotic challenge, generally reverts to the original state once the inducing signal is removed (Fernández et al. 2011; Joon-Hee 2019; Motta et al. 2015; Salimiyan Rizi et al. 2018). Adaptive resistance seems to be the result of modulations in gene expression as a response to environmental changes. Rather than being caused by genetic alterations, which usually produce irreversible phenotypes, adaptive resistance is possibly the result of epigenetic changes. Specifcally, it has been suggested that DNA methylation by the DAM methylase could be responsible for the presence of diferent gene expression profles in a bacterial population and could provide the heterogeneity and epigenetic heredity of gene expression essential for adaptive resistance to occur (Motta et al. 2015; Salimiyan Rizi et al. 2018). In particular, modulation of the expression of efux pumps and porins have been implicated in the emergence of adaptive resistance (Motta et al. 2015). 28 Journal of Molecular Evolution (2020) 88:26–40 1 3 The phenomenon of adaptive resistance may be responsible for the differences observed when comparing the in vitro and in vivo efectiveness of an antibiotic and could be involved in the clinical failure of antibiotic treatments. Moreover, the increase in resistance in response to environmental stimuli may not completely revert upon removal of the stimulus leading to a gradual increase in MIC over time (Fernández et al. 2011). Finally, it has been proposed that the ability of bacterial populations to proliferate in the presence of sub-inhibitory levels of antibiotics through adaptive resistance may eventually allow for more efective and permanent mechanisms of resistance to develop (Fernández et al. 2011; Salimiyan Rizi et al. 2018). Mechanisms of Antibiotic Resistance Resistance to antibiotics is typically the result of antibiotic destruction or modifcation, target alterations (target replacement, target site mutations, target site enzymatic alterations, target site protection, target overproduction or target bypass), and reduced antibiotic accumulation due to either decreased permeability and/or increased efux. Alternatively, antibiotic resistance can be the result of a global adaptation of the bacterial cell (Table 1). We discuss the main antibiotic resistance mechanisms with their clinically relevant impact. Antibiotic Destruction β-Lactamases are the best example of antibiotic resistance mediated by the destruction of the antibiotic molecule. These enzymes destroy the amide bond of the β-lactam ring essentially rendering the antimicrobial inefective. The frst β-lactamases were described in 1940 (Abraham and Chain 1940), 1 year before penicillin was introduced into clinical practice. More than 1000 β-lactamases have been reported up to date produced by diverse bacteria (www.lahey.org/ studies) and are considered to be the most common resistance mechanism leading to β-lactam resistance among Gram-negative bacteria. Genes encoding β-lactamases can be found in the chromosome or in mobile genetic elements (MGEs), which has facilitated their dissemination among bacteria. TEM-1, a plasmid-encoded β-lactamase, was described in Gram-negative bacteria in the 1960s. From then on, the introduction of new β-lactams was followed by the identifcation of new β-lactamases capable of destroying the novel compound. The introduction of third-generation cephalosporins, for example, in the early 1980s was quickly followed by the identifcation of plasmid-encoded β-lactamases capable of hydrolyzing third-generation cephalosporins (Extended-Spectrum β-Lactamases-ESBLs) in 1983 (Knothe et al. 1983). β-lactamase groups of great clinical importance in Gram-negative bacteria include ESBLs (enzymes conferring resistance to penicillins, frst-, second-, third-generation cephalosporins, and aztreonam but not cephamycins or carbapenems and which are inhibited by β-lactamase inhibitors) (Paterson and Bonomo 2005), AmpC enzymes (enzymes conferring resistance to penicillins, frst-, second-, third-generation cephalosporins, aztreonam, and cephamycins but not carbapenems and which are not inhibited by β-lactamase inhibitors) (Jacoby 2009) and carbapenemases (a diverse group of enzymes conferring carbapenem resistance with many conferring resistance to almost all hydrolyzable β-lactams) (Queenan and Bush 2007). Antibiotic Modifcation Enzymatic modifcation of the antibiotic molecule is the commonest mechanism of clinically relevant aminoglycoside resistance. Aminoglycoside modifying enzymes (AMEs) mediate aminoglycoside acetylation, phosphorylation, or adenylation with the resulting modifed antibiotic having a decreased avidity for its target. The genes encoding AMEs are usually located in MGEs enabling them to efciently disseminate among bacteria. As a result, virtually all medically important bacteria can demonstrate aminoglycoside resistance through this mechanism (Ramirez and Tolmasky 2010). Enzymatic acetylation of the antibiotic molecule is the commonest mechanism of chloramphenicol resistance. Many chloramphenicol acetyltransferases (CATs) have been described in a wide range of bacterial species (Schwarz et al. 2004). Modifcations of Antibiotic‑Activating Enzymes Activation of nitrofurantoin by bacterial reductases resulting in the formation of toxic intermediate compounds is required for nitrofurantoin antimicrobial activity. Mutations in the nitroreductase genes nfsA and nfsB comprise the principal mechanism of nitrofurantoin resistance (Osei Sekyere 2018; Whiteway et al. 1998). Mutations in the ribE gene have also been implicated in nitrofurantoin resistance. The ribE gene encodes a lumazine synthase, an enzyme required for the biosynthesis of ribofavin (an important co-factor of nfsA and nfsB) (Osei Sekyere 2018). Target Replacement or Target Bypass Replacement of the bacterial Penicillin-Binding Proteins (PBP) is the mechanism underlying β-lactam resistance in Streptococcus pneumoniae and methicillin resistance in Staphylococcus aureus. β-lactam resistance among Streptococcus pneumoniae is the result of the production of mosaic PBP genes. These genes are produced by the recombination of native DNA and foreign DNA originating from β-lactam-resistant Journal of Molecular Evolution (2020) 88:26–40 29 1 3 Table 1 Examples of major antimicrobial resistance mechanisms with the respective antimicrobials and bacteria involved Antibiotic resistance mechanism Classical examples Antibiotics afected Examples of bacteria utilizing this mechanism Antibiotic destruction β lactamases Penicillinases Penicillins, narrow spectrum cephalosporins Staphylococcus aureus Neisseria Gonorrhoeae Haemophilus Infuenzae Enterobacteriaceae Extended-Spectrum β lactamases Penicillins, frst-, second-, third-generation cephalosporins, and aztreonam Enterobacteriaceae Pseudomonas aeruginosa AmpC enzymes Penicillins, frst-, second-, third-generation cephalosporins, aztreonam, and cephamycins Enterobacteriaceae Acinetobacter spp. Pseudomonas spp. Carbapenemases Carbapenems and almost all hydrolyzable β-lactams Enterobacteriaceae Pseudomonas aeruginosa Stenotrophomonas maltophilia Acinetobacter spp. Antibiotic modifcation Aminoglycoside Modifying Enzymes (AMEs) Aminoglycosides A wide range of bacteria Chloramphenicol acetyltransferases (CATs) Chloramphenicol A wide range of bacteria Modifcations of antibiotic-activating enzymes Mutations in the nitroreductase genes nfsA and nfsB Nitrofurantoin Enterobacteriaceae Target replacement or bypass Penicillin-Binding Protein (PBP) replacement β lactams Streptococcus pneumoniae Acquisition of a novel Penicillin-Binding Protein (PBP) β lactams Staphylococcus aureus Replacement of the terminal d-Alanine d-Alanine moiety of the peptidoglycan precursors Glycopeptides Enterococci Staphylococcus aureus (rarely) Acquisition of a novel dihydrofolate reductase Trimethoprim Gram-negative bacteria Staphylococci Listeria monocytogenes Acquisition of a novel dihydropteroate synthase Sulfonamides Gram-negative bacteria Utilization of exogenous folinic acid Trimethoprim Sulfonamides Enterococci 30 Journal of Molecular Evolution (2020) 88:26–40 1 3Table 1 (continued) Antibiotic resistance mechanism Classical examples Antibiotics afected Examples of bacteria utilizing this mechanism Target site alteration (mutation or enzymatic alteration) Mutations of the 23SrRNA Linezolid Enterococci Staphylococcus aureus Macrolides Lincosamides Streptogramin B A wide range of bacteria Mutations in the bacterial Gyrase or Topoisomerase IV Quinolones A wide range of bacteria Mutations in the RNA Polymerase β subunit Rifampicin A wide range of bacteria Mutational or recombinational changes in the Dihydrofolate Reductase gene Trimethoprim A wide range of bacteria Mutational or recombinational changes in the dihydropteroate synthase gene Sulfonamides A wide range of bacteria Methylation of the 23SrRNA by erm genes Macrolides Lincosamides Streptogramin B A wide range of bacteria Methylation of the 23SrRNA by the cfr gene Linezolid Staphylococcus aureus Chloramphenicol Clindamycin Staphylococci Target site protection Ribosomal Protection Proteins (RPPs) Tetracyclines A wide range of bacteria Qnr proteins Quinolones A wide range of bacteria Target overproduction Overproduction of Dihydrofolate Reductase Trimethoprim Escherichia Coli Decreased permeability of the bacterial outer membrane Mutations afecting the expression or function of porins Hydrophilic antibiotics (e.g., β lactams, quinolones, tetracyclines, chloramphenicol) Gram-negative bacteria Antibiotic efux Multidrug efux pumps A wide range of antibiotics A wide range of bacteria Substrate-specifc efux pumps Tetracyclines A wide range of bacteria Macrolides Streptococci and other gram-positive organisms Chloramphenicol A wide range of bacteria Global cell adaptations Mutations in genes encoding regulatory systems controlling cell envelope homeostasis and genes involved in phospholipid metabolism Daptomycin Enterococci Mutations in genes involved in key cell membrane events and modifcations of the cell wall Daptomycin Staphylococcus aureus Mutations in genes forming part of regulatory systems controlling cell envelope homeostasis resulting in cell wall thickening Intermediate susceptibility to glycopeptides Staphylococcus aureus Journal of Molecular Evolution (2020) 88:26–40 33 1 3 Evolution of Antimicrobial Resistance The Ancient and Diverse Resistome: A Potential Source of Clinically Relevant Resistance Genes In recent times, we have seen antimicrobial resistance rapidly emerge at a global scale and spread from one country to the other faster than previously thought. Superbugs and multidrug-resistant bacteria are endemic in many parts of the world. There is no question that the widespread use, overuse, and misuse of antimicrobials during the last 80 years have been associated with the explosion of antimicrobial resistance. On the other hand, the molecular pathways behind the emergence of antimicrobial resistance in bacteria have been present since ancient times (Dcosta et al. 2011). Commensals and soil bacteria have been capable of producing compounds and small molecules with antimicrobial potential throughout evolution. For example, the emergence of the biosynthetic pathway for erythromycin may date as back as 800 million years (Baltz 2006). What is recently better understood is that these inhibitors did not solely evolve to help bacteria survive but also served other functions like cell signaling or contributed in natural degradation processes, quorum sensing, and bioflm formation (Davies et al. 2006; Sengupta et al. 2013). Moreover, we know that bacterial penicillinases were present before the widespread use of penicillin, while genes encoding β-lactamases have been recovered from very remote environmental habitats (Allen et al. 2009; Bhullar et al. 2012) and from ancient bacteria (Dcosta et al. 2011; Wright 2007). Likewise, it has been found that antibiotic resistance genes exist in the gut of people who live in isolated areas and who have been minimally or never exposed to antimicrobials (Bartoloni et al. 2009; Pallecchi et al. 2007). Thus, there is an increasing body of evidence showing that resistance genes are naturally occurring and in abundance in microbial populations (Dantas et al. 2008; D’Costa et al. 2006). The antimicrobial resistance genes found in diferent bacterial species in nature comprise the environmental resistome (Wright 2007). Sequencing and genomic analysis of extensive bacterial libraries (D’Costa 2006; Dantas et al. 2008) has led to the creation of large databases that list thousands of potential resistance genes (Lakin et al. 2017; McArthur et al. 2013). The resistome consists of known and likely novel genetic resistance determinants, which carry the potential of causing clinically relevant resistance, if successfully transferred and/or expressed in pathogens causing human disease. Species like soil bacteria, which produce antimicrobial products, are obviously intrinsically resistant to those molecules. For example, most antibiotic-producing actinomycetes possess genes encoding resistance to the compounds that they produce (Ogawara et al. 1999). Such resistance mechanisms include genes encoding for β-lactamase production or efux pumps (Cantón 2009; Forsman et al. 2009; Piddock 2006a, b). Some of these resistance mechanisms have been the ancestors of those found in clinically relevant antibiotic-resistant pathogens, as evidenced by their similar biochemical and genetic profles (Benveniste and Davies 1973; Cantón 2009). In addition, old or novel β-lactamases and other resistance determinants can arise as new threats under conditions that will facilitate their transfer to pathogenic bacteria or their enhanced expression. As Kluyvera spp were found to be the source of CTX-M genes, species like Streptomyces known to produce β-lactamases could be the source of clinically important resistance genes (Livermore et al. 2007; Rossolini et al. 2008). Interestingly, Kluyvera spp do not exhibit intrinsic resistance to extended-spectrum β-lactams nor produce antimicrobial compounds (Cantón 2009). It seems that the blaCTX-M genes in Kluyvera spp have originated from a common ancestor, which was incorporated after horizontal transfer into the Kluyvera chromosome, in ancient times (Rossolini et al. 2008). Other examples of such genetic transfers have been documented for genes encoding ribosomal methylases afecting aminoglycosides (armA, rtmB), methyltransferases afecting linezolid (cfr), or plasmid-mediated efux pumps conferring low-level fuoroquinolone resistance (qepA), all of which were associated with antibiotic-producing bacteria (Cantón 2009). However, we need more phylogenetic studies and metagenomics data in order to defne the exact role of the environmental resistome in the emergence of antimicrobial resistance in human and animal pathogens. Emergence of Antimicrobial Resistance: Random Events, Genetic Exchange, and Selection Pressure Resistance in bacteria or bacterial communities arises via two major mechanisms, either pick-up/transfer of resistance genes from other bacteria or gene mutation of pre-existing or acquired genes. Strong selection of these genetic events is believed to be enhanced in the presence of antimicrobials (Cantón 2009; Gillings et al. 2017). Under inhibitory and sub-inhibitory concentrations of antimicrobials, bacteria that can survive will be selected (Davies and Davies 2010; Gillings et al. 2017). Nonetheless, some antibiotics can modulate the expression of virulence factors or upregulate genes involved in the SOS response and potentially contribute to increased mutation frequency, via the latter (Sengupta et al. 2013). It has been hypothesized that in the antibiotic era, naturally occurring selection occurred and spread more rapidly compared to the pre-antibiotic era (Cantón 2009; Davies and Davies 2010; Gillings et al. 2017). This can occur either in the environment or within the host. 34 Journal of Molecular Evolution (2020) 88:26–40 1 3 The emergence of a multitude of antimicrobial-resistant microbes and their now established presence not only in the clinical setting but also in the environment has been facilitated by the widespread use of antibiotics in human health, agriculture, aquaculture, animal farming, veterinary medicine, pest control, and pharmaceutical industry. Nonetheless, it is well documented that increased antibiotic use is correlated with higher resistance rates, as countries with low rates of antimicrobial resistance also report lower rates of antimicrobial consumption (Costelloe et al. 2010). Pathogens can exchange pathogenic, virulence, and resistance elements. Transmission by conjugation occurs extensively both in nature and within the host, most abundantly in the intestinal tract (Sommer et al. 2010). Horizontal gene transfer is most commonly mediated through plasmids, which have a key role in the spread of resistance between bacterial strains (Davies and Davies 2010; Norman et al. 2009). For example, resistance genes, which encode modifed β-lactamases, can be transferred rapidly and efciently horizontally. One of the original types of β-lactamases known to confer resistance to gram-negative bacteria is TEM. However, nowadays, plenty of plasmid-encoded TEM enzymes, stemming from random mutations (rapid radiation), have occurred and circulate globally (Gniadkowski 2008). Also, extended-spectrum β-lactamases (ESBL) now encoded by more than 100 CTX-M variant genes have shown an enormous ability to transmit and spread all over the world (Rossolini et al. 2008). Interestingly, however, plasmid sequencing of pre-antibiotic era bacterial pathogens has shown that resistance determinants on plasmids were not common (Datta and Hughes 1983). Resistance acquisition through genetic exchange via plasmids, transposons, and integrons, is favored in dense communities like sewage treatment plants, considered as “hotspots” for bacterial genetic exchange. Plasmids carrying multiple resistant genes have been recovered from samples taken from sewage (Schlüter et al. 2007), where indigenous bacteria and those derived from humans and animals coexist. For example, Aeromonas cultured from aquaculture environments has been shown to carry multiple resistance genes on plasmids and integrons (Penders and Stobberingh 2008). The human gut is considered another hotspot for such genetic exchanges between pathogens and commensals (Sommer et al. 2010). In recent years, there has been an increasing interest on the contribution of integrons in bacterial genome evolution, due to their role in promoting genetic diversity in bacteria (Boucher et al. 2007; Mazel 2006). Integrons were frst described in 1987 (Stokes and Hall 1989) and are found on the chromosomes of approximately 15% of bacterial species (Boucher et al. 2007; Mazel 2006). However, it was later demonstrated that they were linked to plasmidmediated resistance encountered in Shigella in the 1950s in Japan (Liebert et al. 1999). The main role of integrons is gene cassette capture followed by recombination into an attachment site (a process catalyzed by integrase) and gene expression via activation of the SOS system. Integrons are a major source of transferable resistance determinants, both in Gram-negative and Gram-positive bacteria (Gillings 2014). Especially class 1 integrons are widely present in pathogens and gut commensals and overall, they have accumulated over 130 gene cassettes conferring resistance to most antibiotic classes (Partridge et al. 2009). Another important evolutionary mechanism exhibited by bacteria is direct DNA acquisition from the environment, like, for example, in Acinetobacter spp, an organism that has evolved rapidly accumulating, in some cases, more than 20 genomic islands encoding antibiotic resistance determinants and virulence functions (Barbe et al. 2004). In addition, development of resistance may be facilitated in mixed bacterial communities, like polymicrobial infections or bioflms, due to cell–cell fusion, larger populations with lower rates of turnover, and elevated mutation rates (Brockhurst et al. 2019; Drifeld et al. 2008). Lastly, physiological tolerance to antibiotics can allow or precede the emergence of resistant phenotypes (Levin-Reisman et al. 2017). Whole genome sequencing can help tremendously in reconstructing the evolutionary history of resistance in bacterial species. For example, using WGS and phylogenetic analysis of the frst MRSA isolates, scientists were able to discover that the early MRSA lineage arose in the mid-1940s (long before the use of methicillin) after the acquisition of an ancestral type I SCCmec element. Therefore, contrary to what was previously thought, the driver for MRSA evolution was not methicillin but the widespread use of frst-generation β-lactams. Such data could inform the assessment of evolutionary risk and the design of novel antimicrobial therapies (Brockhurst et al. 2019; Harkins et al. 2017). Resistance genes can cross species and similar resistance genes have been found in humans and animals. Whole genome sequencing of certain strain lineages like S. aureus CC398 has provided evidence of many livestockto-human jumps and less frequently, human-to-livestock jumps during its evolutionary history (Ward et al. 2014). In another landmark study and contrary to what was previously believed, in 2015, Liu et al. (Liu et al. 2016) frst described transferable colistin resistance in E. coli and K. pneumoniae isolates from animals and humans, mediated by the mcr-1 gene (mobile colistin resistance), located in a plasmid. Since then, more mcr variants have been discovered, in several bacteria in diferent countries (Dalmolin et al. 2018). These examples highlight the importance of a one-health approach in trying to address the antimicrobial resistance crisis. Journal of Molecular Evolution (2020) 88:26–40 35 1 3 Drivers of Antimicrobial Resistance Persistence Although it is indisputable that the use of antibiotics in clinical and veterinary practices as well as animal husbandry is strongly correlated with the development and spread of antibiotic resistance, it is less evident that reducing the use of antibiotics will result in signifcant reductions in antibiotic resistance. There are, of course, several reports of antibiotic resistance decreases as a result of a reduction in antibiotic use such as the decrease in penicillin resistance in Streptococcus pneumoniae in Hungary following a drastic reduction in the prescription of penicillin (Nowak 1994). However, there are also reports of antibiotic resistance persistence despite drastic reductions in antibiotic use. No signifcant reduction of resistance was observed following drastic reductions in the use of trimethoprim and sulphonamides in Sweden and the United Kingdom, respectively (Andersson et al. 2009; Enne et al. 2001). A possible explanation could be that antibiotic exposure extends beyond therapeutic use in humans, which accounts for less than half of the total antibiotic consumption (Davies and Davies 2010). It does, therefore, seem that antibiotic selection pressure may not be the only driver of resistance. The acquisition of antibiotic resistance mechanisms is usually associated with a ftness cost for the bacterial cell. Resistance due to chromosomal mutations often involves modifcation of essential bacterial genes while resistance due to the acquisition of plasmids imposes a burden on the host cell due to the metabolic load of plasmid replication and the expression of plasmid genes, disruptions in the expression of host genes, and other metabolic efects. As a result, antibiotic-resistant bacteria tend to have a reduced growth rate and competitive ability compared to their susceptible counterparts (Carroll and Wong 2018; Vogwill and Maclean 2015). One would, therefore, expect that the absence of antibiotic selection pressure would lead to a reduction in antibiotic resistance with sensitive bacteria displacing their less ft resistant counterparts. However, antibiotic resistance genes seem to persist for long periods of time in the absence of antibiotic selection pressure. Antibiotic resistance stabilization in bacterial populations and persistence in environments with reduced antibiotic use can occur via the following mechanisms. Fitness Restoring Compensatory Mutations Compensatory mutations in other loci, which restore the ftness of antibiotic-resistant bacteria, can allow the maintenance of antibiotic-resistant bacteria even in the absence of antibiotic selection. In vitro and in vivo experiments with antibiotic-resistant bacteria have shown that bacterial evolution in the absence of antibiotics is more likely to result in compensatory mutations improving the ftness of antibiotic-resistant bacteria rather than in reversion to highly ft antibiotic-sensitive bacteria (Levin et al. 2000; Schrag et al. 1997). In the case of conjugative plasmids, these compensatory adaptive mutations have been described to occur on the bacterial chromosome, the plasmid or both (Dahlberg and Chao 2003; Harrison et al. 2016). The Occurrence of Fitness Cost‑Free or Fitness Increasing Resistance Mechanisms Although resistance mechanisms are usually associated with a ftness cost, this is by no means always the case. There are reports of antibiotic resistance plasmid acquisitions which are either ftness cost-free (Carroll and Wong 2018; Enne et al. 2005) or are associated with an increase in the ftness of the bacterium (Carroll and Wong 2018; Enne et al. 2004). The ftness efect of a plasmid does not seem to be constant but instead is infuenced by the host genetic background (Carroll and Wong 2018; Di Luca et al. 2017). Fitness enhancing chromosomally encoded antibiotic resistance mutations has also been described (Luo et al. 2005). Genetic Linkage and Co‑selection with Other Genes Genetic linkage of multiple antibiotic resistance genes allows for their co-selection. Consequently, reducing the use of one antibiotic may not result in the expected reduction in resistance to this agent if the use of other antibiotics continues to be high (Andersson and Hughes 2011). This is particularly important for plasmid-encoded antibiotic resistance since, as mentioned previously, multiple resistance genes often accumulate on a single plasmid. Alternatively, an antibiotic resistance gene can be co-selected with other genes such those encoding virulence factors (Andersson and Hughes 2011; Salyers and Amábile-Cuevas 1997) or genes encoding resistance to biocides and metals (Pal et al. 2015). Plasmid Persistence Mechanisms Apart from the mechanisms mentioned above which can result in the persistence of both chromosomally encoded and plasmid-encoded antibiotic resistance mechanisms, additional mechanisms specifcally ensuring plasmid persistence exist. Plasmid stabilization systems include the multimer resolution system, active partitioning, and various types of post-segregational killing systems (which kill individual cells lacking the plasmid) (Andersson and Hughes 2011; ANRV337-PU29-10 ARI 10 March 2008 19:57 Industrial Food Animal Production, Antimicrobial Resistance, and Human Health Ellen K. Silbergeld, Jay Graham, and Lance B. Price Bloomberg School of Public Health, Johns Hopkins University, Baltimore, Maryland 21205; email: [email protected], [email protected], [email protected] Annu. Rev. Public Health 2008. 29:151–69 The Annual Review of Public Health is online at http://publhealth.annualreviews.org This article’s doi: 10.1146/annurev.publhealth.29.020907.090904 Copyright c 2008 by Annual Reviews. All rights reserved 0163-7525/08/0421-0151$20.00 Key Words bacteria, agriculture, drug-resistant pathogens, infectious disease, horizontal gene transfer, poultry, environment Abstract Antimicrobial resistance is a major public health crisis, eroding the discovery of antimicrobials and their application to clinical medicine. There is a general lack of knowledge of the importance of agricultural antimicrobial use as a factor in antimicrobial resistance even among experts in medicine and public health. This review focuses on agricultural antimicrobial drug use as a major driver of antimicrobial resistance worldwide for four reasons: It is the largest use of antimicrobials worldwide; much of the use of antimicrobials in agriculture results in subtherapeutic exposures of bacteria; drugs of every important clinical class are utilized in agriculture; and human populations are exposed to antimicrobial-resistant pathogens via consumption of animal products as well as through widespread release into the environment. 151 Annu. Rev. Public Health 2008.29:151-169. Downloaded from www.annualreviews.org Access provided by University of California – Riverside on 11/02/20. For personal use only. ANRV337-PU29-10 ARI 10 March 2008 19:57 Antimicrobial resistance: the property of microbes to thwart the toxicity of agents designed to control them Reservoirs of resistance: the collection of genetic information, encoding resistance, available to microbial communities through mechanisms of horizontal gene transfer INTRODUCTION: ANTIMICROBIAL RESISTANCE AND AGRICULTURE Antimicrobial resistance is an increasing crisis in clinical and veterinary medicine worldwide. Although antimicrobial resistance is an inevitable consequence of the evolutionary adaptation of microbes, human use and misuse of antimicrobial drugs have driven the increasingly rapid and prevalent emergence of resistance in a range of pathogenic and commensal organisms. This review focuses on agricultural antimicrobial drug use as a major driver of antimicrobial resistance worldwide for the following reasons: First, the largest use of antimicrobials worldwide occurs in the production of animals for human consumption of meat, milk, and eggs; second, antimicrobials are used as additives in animal feeds; third, drugs from almost every mechanistic class of clinically valuable agents are utilized in agriculture; and fourth, this use results in human exposure to antimicrobial-resistant pathogens via food and through widespread release into the environment. Antimicrobial resistance is a major public health crisis (40), threatening the return of untreatable infections on a massive scale. Antimicrobial-resistant bacterial infections now account for many emerging infectious diseases worldwide (17, 54). In some pathogens, selection for resistance also results in increased virulence (73). There is a general lack of knowledge of the importance of agricultural antimicrobial use as a factor in antimicrobial resistance even among experts in medicine and public health. A recent analysis of the problem of antimicrobial resistance (36) devotes less than one page to the issue of agricultural antimicrobial use. Although clinical issues are not unimportant, the scale of clinical use and misuse is dwarfed by the magnitude of the largely unregulated use of antimicrobials in agriculture. Moreover, the increasing problems of food-borne drug-resistant infections are seldom linked to their origin in food animal production, which inhibits the effectiveness of public health policies to prevent food borne illness. Most importantly, the problem is often conceptualized in terms of resistance to specific antimicrobials in pathogens of clinical importance, rather than ecologically in terms of reservoirs of resistance genes that may flow across the microbial ecosystem. The goal of this review is to elucidate these issues through a critical assessment of underlying biological mechanisms involved in the evolution and interecosystem spread of antimicrobial resistance from agriculture, and of the evidence associating agricultural use with the increasing prevalence of antimicrobialresistant pathogens in food and the environment, as well as of resistant infections in human populations. As discussed below, evidence indicates that agricultural use of antimicrobials in feeds has compromised the efficacy of most antimicrobials used in the United States and throughout the world. For this reason, since 1997 the World Health Organization, together with the Food and Agriculture Organization and the International Organization for Epizootics, has consistently recommended restrictions on nontherapeutic uses of antimicrobials in agriculture, reporting on all antimicrobial uses, and veterinary oversight on the use of antimicrobials in food animals (85). USE OF ANTIMICROBIALS IN AGRICULTURE Antimicrobials are utilized in agriculture for veterinary medicine, as feed additives, and as biocides (in crop and fruit production). The major agricultural use of antimicrobials is in the production of poultry, swine, and cattle, but antimicrobials are also used in aquaculture (7) and there are limited uses for it in plants (44). These practices, which are relatively recent in agronomy, have been accompanied by an organizational transformation of agriculture over the past 60 years. It is important to understand how these changes have substantially altered the relationship between 152 Silbergeld · Graham · Price Annu. Rev. Public Health 2008.29:151-169. Downloaded from www.annualreviews.org Access provided by University of California – Riverside on 11/02/20. For personal use only. ANRV337-PU29-10 ARI 10 March 2008 19:57 humans and animals in terms of transmission of infectious disease through food and other pathways. Intensive or industrial food animal production (IFAP) originated in the United States in the late 1930s (22). This has resulted in an integrated model of production, where large corporations control most aspects of animal husbandry, processing of animals into food products, and sales to the consumer market (43). Two aspects of IFAP have introduced new pathogen risks to both animal and human health: the dense confinement of large numbers of animals, and new formulations of animal feeds. Confinement of large populations of animals in buildings or feedlots is a characteristic of IFAP; these facilities are often referred to as concentrated animal feeding operations (CAFOs), depending upon their size. Confinement has several impacts on pathogen risks for animals as well as humans in that contact of large numbers of susceptible hosts facilitates the exchange and evolution of pathogens (61). In general, risks of zoonotic disease are greatly intensified by an increased scale of animal husbandry (18). Most importantly, confined animal populations are unavoidably exposed to their waste. Poultry are housed with their waste, while hogs are housed on top of waste pits. These conditions are illustrated in Figure 1, (http://photogallery.nrcs.usda.gov). CAFOs are comparable to poorly run hospitals, where everyone gets antibiotics, patients lie in unchanged beds, hygiene is nonexistent, infections and re-infections are rife, waste is thrown out the window, and visitors enter and leave at will. Finally, because these large numbers of animals produce large amounts of waste, which are largely untreated prior to land disposal, there are substantial environmental pathways of release and exposure. The formulation of feeds also influences pathogen risks. The feeds supplied to confined animal populations are significantly different from the foraged feeds traditionally Industrial food animal production (IFAP): a mode of animal husbandry characterized by high density and confinement of animals (including poultry, pigs, cattle, and aquatic species) raised for human food available to poultry, swine, or cattle (with relatively minimal supplementation by minerals or other substances). Modern animal feeds are formulated with proteins and fats from crops (largely corn and soybean derived), animal fats and proteins (recycled through rendering), additions of industrial waste streams, animal waste, and antimicrobials (reviewed in Reference 64). This latter innovation, which began more than 50 years ago in the United States (51), has introduced a major driver for the selection and dissemination of antimicrobial resistance in bacteria Until recently, there was no examination of the actual effect of antimicrobial feed additives on food animal production at the commercial scale. By using data published by the Perdue Company (16), Graham et al. (22) found a very small positive impact of antimicrobial feed additives such that the marginal benefit did not offset the cost of purchasing antimicrobials for addition to feeds. Moreover, the assumed benefits of antimicrobials as growth promoters can be achieved by improved cleanliness of animal houses (16, 46). A wide range of antimicrobial drugs are permitted for use in food animal production in the United States and many other countries (65). As shown in Table 1, these drugs represent all the major classes of clinically important antimicrobials, from penicillin to third-generation cephalosporin compounds. In some cases, new drugs were licensed for agricultural use in advance of approvals for clinical use. In the case of quinupristindalfopristin (virginiamycin), this decision by the Food and Drug Administration resulted in the emergence of resistance in human isolates prior to eventual clinical registration (33), thus demonstrating how feed additive use can compromise the potential utility of a new tool in fighting infectious disease in humans. For existing drugs, Smith et al. (70) calculated that agricultural use can significantly shorten the useful life of antimicrobials for combating human or animal disease. www.annualreviews.org • Food Animal Production and Human Health 153 Annu. Rev. Public Health 2008.29:151-169. Downloaded from www.annualreviews.org Access provided by University of California – Riverside on 11/02/20. For personal use only. 1612 28 SEPTEMBER 2012 VOL 337 SCIENCE www.sciencemag.org PERSPECTIVES is proportional to the square of the angular velocity Ω and which acts outward from the axis of rotation to make the equator bulge; the other is the aspherical deviation ∆Φ of the gravitational potential produced by the centrifugal force distorting the inside of the Sun, principally at mid-radii (see the fi gure). From the angular velocity of the visible surface ( 4), the former is determined to be ∆Ω ~ 8.4 (±0.2) × 10−6. It was then expected that ∆Φ could be obtained by simply subtracting ∆Ω from ∆v . Had the entire anomalous precession been due to a solar oblateness, ∆Φ would have dominated the full oblateness, and the procedure would have been viable. But general relativity indicated that ∆Φ is much smaller, and helioseismic observations ( 5) indicate that it is only 3.3 × 10−7 , just 4% of ∆Ω, thereby implying that ∆v should be 8.7 × 10−6 . The fi rst of the modern observations were made serendipitously ( 1) using the guiding sensor for the Reuven Ramaty High-Energy Solar Spectroscopic Imager (RHESSI) ( 6). A rapidly rotating optical image of the solar limb was Fourier-analyzed with respect to the angle about the line of sight to estimate the quadrupolar component of the limb displacement. A major problem with the measurement, as with any other limb-shape measurement, is brightness contamination by sunspots and magnetically generated excess emission. In an attempt to eliminate the detritus, a magnetic-activity proxy was adopted to reject suspect data ( 7). The outcome was ∆v = 8.34 (±0.15) × 10−6 . Kuhn et al. present an analysis of limbbrightness data in a photospheric Fe spectral line obtained from HMI. The limb-darkening function was measured as a function of the angular orientation of the Sun’s image at times when the spacecraft was rotated for calibration purposes about its pointing axis. Magnetic contamination was removed by rejecting limb-position data associated with brightness above some threshold that had been judged to yield a robust outcome. Detailed analysis ( 8) then yielded ∆v = 7.56 (±0.40) × 10−6 . Note that the uncertainties in the values of ∆v are not signifi cantly smaller than ∆Φ. Therefore, subtracting ∆Ω from ∆v is evidently not a viable procedure for determining ∆Φ ( 9, 10). More startling, however, is that Kuhn et al. found ∆v to be some 10% lower than the value of ∆Ω expected from the surface rotation. Therefore, if their observations are correct, something else other than rotation must be affecting the limb. Until that is explained and quantifi ed precisely, solar limb shape cannot provide a means to test gravity; helioseismology is, and will remain, the only accurate tool to do so. The new oblateness measurements beg explanation. An expected polar temperature excess comes to mind ( 11); however, Kuhn’s ( 12) estimate of the excess is just 1 to 2 K, which is grossly insuffi cient to explain the discrepancy. Alternatively, one could wonder whether polar support is boosted by an intense large-scale surface magnetic fi eld; might not the intensity of the fi eld demanded for that be plausible if the spatial variation in the differential rotation requires forces, perhaps magnetic, of suffi cient strength to produce a shape distortion of similar magnitude to that produced directly by the differential rotation itself? That is not necessarily so, as any child in a playground who has spun up a merry-go-round with ease but then had diffi culty holding on against the resulting centrifugal force well knows. Even if a magnetic hill were present, the surface material would surely drain away. What other possibility is left? Turbulent stresses from convection, perhaps? These newest data have left us with an intriguing new conundrum in solar physics: Why does the Sun appear to be so round? References and Notes 1. M. D. Fivian, H. S. Hudson, R. P. Lin, H. J. Zahid, Science 322, 560 (2008). 2. J. R. Kuhn, R. Bush, M. Emilio, I. F. Scholl, Science 337, 1638 (2012); 10.1126/science.1223231. 3. P. H. Scherrer et al., Sol. Phys. 275, 207 (2012). 4. J. G. Beck, Sol. Phys. 191, 47 (2000). 5. F. P. Pijpers, Mon. Not. R. Astron. Soc. 297, L76 (1998). 6. M. D. Fivian, R. Henneck, A. Zehnder, in Innovative Telescopes and Instrumentation for Solar Astrophysics, S. L. Keil, S. V. Avakyan, Eds., (SPIE, Waikoloa, HI, 2003), p. 60. 7. J.-P. Delaboudinière et al., Sol. Phys. 162, 291 (1995). 8. Kuhn et al. fi nd no evidence for a variation of ∆v associated with the solar cycle; the gravitational oblateness ∆Φ varies by less than 0.04% ( 13). Yet solar-cycle variations in more convoluted distortions in the gravitational equipotentials and perhaps the surface shape appear to occur. It is not clear whether these phenomena are causally related. 9. Fivian et al. ( 1) adopted a value for ∆Ω quoted by Dicke ( 10), who had evaluated it using an inaccurate representation of the Sun’s surface angular velocity Ωs ; that estimate of ∆Ω is actually lower than ∆v by approximately ∆Φ. 10. R. H. Dicke, Astrophys. J. 159, 1 (1970). 11. J. L. Tassoul, Stellar Rotation (Cambridge Univ. Press, Cambridge, 2000). 12. J. R. Kuhn, in Encyclopedia of Astronomy and Astrophysics, P. Murdin, Ed. (Institute of Physics, Bristol, UK, 2001), article 2245. 13. H. M. Antia, S. M. Chitre, D. O. Gough, Astron. Astrophys. 477, 657 (2008). 14. D. O. Gough, Nature 298, 334 (1982). 10.1126/science.1226988 Rachel Carson’s book Silent Spring, published 50 years ago ( 1), eloquently awoke the public to the manifold dangers for the environment and human health posed by the wanton use of chemical pesticides ( 2). Carson argued that in addition to their many harmful ecological effects, chemical insecticides ultimately undermine sustainable pest management: They kill the parasites and predators that formerly held many pests in check, while the pests themselves become resistant and require ever-higher amounts of sprays for their control. Since the publication of Silent Spring, more than 450 arthropod species have been reported with resistance to one or more pesticides ( 3). Yet over the same period, a paradigm shift in dealing with this global problem has also occurred. Resistance, defined as the heritable decrease in a population’s susceptibility to a toxin to which it is exposed over successive generations, is an example of evolution by natural selection. The intensity of selection can be controlled by varying the insecticide and reducing the frequency and intensity of application. A variety of different chemical classes has been developed, targeting a range of biological targets in the insect. Yet because the number of targets is still limited and new targets are not resistance-proof, prolonging the useful life of existing insecticides by judicious use has increasing priority in their Insecticide Resistance After Silent Spring ECOLOGY David G. Heckel Combating insecticide resistance is a continual challenge for the preservation of both traditional and transgenic crops. Department of Entomology, Max Planck Institute for Chemical Ecology, Hans-Knöll-Str. 8, D-07745 Jena, Germany. E-mail: [email protected] Published byAAAS on October 19, 2015 www.sciencemag.org Downloaded from www.sciencemag.org SCIENCE VOL 337 28 SEPTEMBER 2012 1613 PERSPECTIVES commercialization ( 4). Because pesticide use is diffi cult to control on a global level, resistance management programs must be locally suitable, economically feasible, and voluntarily adopted by growers. Pesticide resistance often results from gene regulatory changes, which lead to an increase in the efficiency of one or more physiological systems used by the insect for detoxification: oxidation, conjugation to hydrophilic compounds, and excretion (see the fi gure). Constitutive up-regulation of these detoxifying enzymes is most common, although gene amplifi cation is another mechanism for increasing the amount of protein available to inactivate the insecticide. In such cases, general inhibitors of classes of detoxifying enzymes may be used to counter the increased detoxifi cation ability. Rarely, a single mutation can confer a novel detoxifying ability, such as the substitution of an aspartate for a glycine in a carboxylesterase of the Australian sheep blowfly, converting it to an organophosphorus hydrolase ( 5). More commonly, single mutations reduce the sensitivity of the insecticide’s biochemical targets, including enzymes and ion channels in the nervous system. In these proteins, which are essential for life, only a very few mutations are compatible with biological function; these mutations are often found in many different species exposed to the same insecticide, representing multiple cases of parallel evolution. Thus, engineering modifi ed insecticides that are more potent on the altered target sites could be of general benefi t. This predictability at the level of target-site mutations is, however, often frustrated by the presence of additional, more diverse detoxifying mechanisms in the same species. A good example is the complexity of resistance in mosquitoes, which are vectors of malaria and other diseases. Gene amplifi cation causing overproduction of organophosphorus-inactivating carboxylesterases has spread worldwide in Culex species ( 6). Some Culex species have evolved further to replace this energetically costly overproduction of protein by mutation and gene duplication of the target, acetylcholinesterase (AChE) ( 7). Bariami et al. recently used microarrays to show that increased expression of a CYP9Jtype P450 and an ABC transporter, partly due to gene amplifi cation, may underlie resistance to pyrethroids in populations of the dengue vector Aedes aegypti ( 8). The aphid Myzus persicae is another serial offender. It fi rst evolved organophosphorus resistance by making up to 80 copies in its genome of a pair of esterase genes, resulting in esterase protein up to 1% of body weight ( 9). This expensive overproduction is switched off by an unknown mechanism when the aphid demethylates these gene copies. Target-site mutations in AChE compensate for this loss; sodium channel mutations additionally confer pyrethroid resistance. The aphid counters the newer neonicotinoid insecticides by multiple duplications of the CYP6CY3 P450 gene ( 10) and a target-site mutation in a subunit of the acetylcholine receptor ( 11); this combination of mechanisms is especially potent. The spider mite Tetranychus urticae is notorious for rapidly evolving resistance, spurring the development of novel chemicals for control. Resistance to binfenazate has been shown to result from four mutations in the mitochondrial DNA that encodes cytochrome b; these mutations occurred at positions that are otherwise completely conserved across eukaryotes, identifying the target site of this new pesticide ( 12). This unusual, maternally inherited resistance responds extremely rapidly to selection in the fi eld. Recently, Van Leeuwen et al. used the mite’s genome sequence to investigate resistance to etoxazole, which inhibits synthesis of the chitinous exoskeleton of arthropods. By selecting a mixed population and tracking frequency changes of more than 700,000 polymorphisms, the authors identified a genome region that contains a mutated chitin synthase gene, with a single amino acid substitution conferring resistance ( 13). Toxicity to nontarget organisms and reduced effectiveness on resistant pests constituted two separate threads of the argument against pesticides in Silent Spring ( 1). Remarkably, these ideas intersect on the genetic model Drosophila melanogaster, which, despite not being a pest, has received enough incidental environmental exposure over the years to develop resistance to several older insecticides. Dieldrin-resistant fl ies from the wild enabled the fi rst identifi cation of the Rdl mutation in the GABAgated chloride channel ( 14), subsequently found in many pest species. One cause of DDT resistance is the insertion of a transposable element in the promoter of CYP6G1 ( 15), leading to overexpression of this P450 enzyme, which detoxifi es DDT. Drosophila later attained even higher DDT resistance by two additional insertions and a gene duplication, revealing an ongoing process of multiple adaptive steps ( 16). To identify A B D C COE P450 ABC Cl O O C N Cl O O O O C N OH Some mechanisms of insecticide resistance. Insects have evolved mechanisms to detoxify, reduce their sensitivity to, or excrete insecticides. Increased detoxifi cation can occur by (A) gene duplication of carboxylesterase (COE), which cleaves the insecticide, or (B) transposon insertion, causing increased transcription of P450, which hydroxylates the insecticide. (C) Point mutations in the target can reduce insecticide binding. (D) Increased transporter activity leads to faster excretion from the cell. Published byAAAS 1614 28 SEPTEMBER 2012 VOL 337 SCIENCE www.sciencemag.org PERSPECTIVES the targets of newer insecticides, Perry et al. have used mutagenesis of Drosophila to create strains resistant to spinosad or neonicotinoids; they pinpointed the acetylcholine receptor subunits that are most sensitive to these toxins ( 17). The goal of reducing the use of chemical insecticides has spurred the search for biologically based alternatives, a strategy encouraged by Carson [chapter 17 in ( 1)]. Insecticidal protein toxins from the bacterium Bacillus thuringiensis (Bt) are now expressed in more than 58 million hectares of transgenic cotton and maize worldwide to deter lepidopteran pests ( 18). When Bt cotton was fi rst introduced in the United States and Australia, government-mandated, industry-implemented resistance management plans were in place. These “high dose/refuge” strategies aimed to slow the process of natural selection, fi rst by ensuring that transgenic plants expressed enough toxin to kill all but the most resistant insects, and second by providing non-Bt crops as “susceptibility refuges” on which Bt-susceptible pests could develop to adulthood and mate with the relatively few survivors from the Bt crop. These strategies to delay resistance are working so far in most cases ( 19). What if they fail? Estimation of the frequency of rare Bt resistance alleles before they become common enough to cause unsustainable crop damage can provide advance warning of developing resistance. Using methods based on the inbreeding of large fi eld samples, Downes and Mahon have detected alleles for resistance to the Cry2Ab toxin at frequencies of 0.5 to 0.9% in two species of bollworms in Australia ( 20). When the resistance gene is known, DNA sequencing can also be used; Zhang et al. have correlated mutations in a 12-cadherin-domain protein with bollworm resistance to the Cry1Ac toxin in China ( 21). Modifi ed Bt toxins have been engineered to circumvent this type of resistance and show promise on other Bt resistance mechanisms as well ( 22). Coexpression of an additional toxin, Vip3A, with a different mode of action has been commercialized to delay pest resistance to transgenic crops; however, the Vip3Aresistant allele frequency is already 2.7% in one pest, which is very high given that there has been no prior exposure to this toxin (23). Forewarned by the long history of insecticide resistance, the deployment of transgenic crops for insect control has incorporated resistance management plans from the beginning. Unfortunately, this has not been the case for transgenic crops engineered for herbicide tolerance. Greatly increased spraying to control weeds in these new crops has led to a rapid rise of herbicide resistance in several weed species ( 24), and agronomists must now follow entomologists in learning the hard lessons of the past 50 years. References 1. R. Carson, Silent Spring (Houghton Miffl in, Boston, 1962). 2. L. J. Guillette Jr., T. Iguchi, Science 337, 1614 (2012). 3. Arthropod Pesticide Resistance Database, www.pesticideresistance.org/. 4. Insecticide Resistance Action Committee, www.iraconline.org/. 5. R. D. Newcomb et al., Proc. Natl. Acad. Sci. U.S.A. 94, 7464 (1997). 6. M. Raymond, A. Callaghan, P. Fort, N. Pasteur, Nature 350, 151 (1991). 7. P. Labbé et al., PLoS Genet. 3, e205 (2007). 8. V. Bariami et al., PLoS Negl. Trop. Dis. 6, e1692 (2012). 9. A. L. Devonshire et al., Philos. Trans. R. Soc. B 353, 1677 (1998). 10. A. M. Puinean et al., PLoS Genet. 6, e1000999 (2010). 11. C. Bass et al., BMC Neurosci. 12, 51 (2011). 12. T. Van Leeuwen et al., Proc. Natl. Acad. Sci. U.S.A. 105, 5980 (2008). 13. T. Van Leeuwen et al., Proc. Natl. Acad. Sci. U.S.A. 109, 4407 (2012). 14. R. H. ffrench-Constant et al., Annu. Rev. Entomol. 45, 449 (2000). 15. P. J. Daborn et al., Science 297, 2253 (2002). 16. J. M. Schmidt et al., PLoS Genet. 6, e1000998 (2010). 17. T. Perry et al., Pestic. Biochem. Physiol. 102, 56 (2012). 18. C. James, Global Status of Commercialized Biotech/GM Crops: 2010 (International Service for the Acquisition of Agri-biotech Applications, Ithaca, NY, 2011). 19. B. E. Tabashnik, A. J. Gassmann, D. W. Crowder, Y. Carriére, Nat. Biotechnol. 26, 199 (2008). 20. S. Downes, R. Mahon, J. Invertebr. Pathol. 110, 281 (2012). 21. H. Zhang et al., Proc. Natl. Acad. Sci. U.S.A. 109, 10275 (2012). 22. B. E. Tabashnik et al., Nat. Biotechnol. 29, 1128 (2011). 23. R. J. Mahon, S. J. Downes, B. James, PLoS ONE 7, e39192 (2012). 24. H. J. Beckie, Pest Manag. Sci. 67, 1037 (2007). Acknowledgments: Supported by the Max-Planck Gesellschaft. 10.1126/science.1226994 Life in a Contaminated World ECOLOGY Louis J. Guillette Jr. 1 and Taisen Iguchi 2 Exposure to pesticides and other chemicals can have complex long-term health effects. U ntil the early 1960s, pesticide use was perceived as a benefi t to agriculture and public health, with few detrimental consequences. This perception changed dramatically with the publication 50 years ago of Rachel Carson’s Silent Spring ( 1). The book was the start of a debate that continues to this day on the relative benefi ts and risks of not just pesticides but all synthetic chemicals. Pesticides are unquestionably benefi cial for food production ( 2), but there is a growing awareness of the risks to human and ecological health associated with their use. Over the past decade, a growing literature ( 3– 6) has examined how early-life exposure to an array of chemical agents, found not only in pesticides but also in personal care products and plastics, can affect human health. The effects on endocrine signaling (and thus endocrine disruption) have been observed in the exposed generation and also in succeeding generations, but the conclusions are not without controversy. “It is ironic to think that man might determine his own future by something so seemingly trivial as the choice of an insect spray” wrote Carson in 1962 [p. 8 in ( 1)]. Although she had no mechanism to explain her observations, it is now well documented that exposure early in embryonic development to commonly used chemicals alters gene expression patterns that can lead to altered health later in life ( 7). But what dose is required to cause an effect? A large literature in the fi elds of endocrinology and general physiology demonstrates not only that different effects can be induced at different doses but also that the mechanisms driving those effects can differ as well ( 7). A report from the Endocrine Society states that different effects should be expected when comparing high- and lowdose regimens of endocrine disruptors ( 3). Studies using acute high-dose exposures may thus be of limited value for predicting what might occur following the chronic low-dose exposures that almost every population on Earth is subjected to today, often at low but detectable concentrations. Early-life exposure to chemicals with endocrine disruption potential has been shown to alter gene expression profi les that 1Department of Obstetrics and Gynecology, Medical University of South Carolina and Hollings Marine Laboratory, 221 Fort Johnson Road, Charleston, SC 29412, USA. 2Okazaki Institute for Integrative Bioscience, National Institute for Basic Biology, Okazaki 444-8787, Japan. E-mail: [email protected] Published byAAAS Pesticide Resistance: Strategies and Tactics for Management Copyright National Academy of Sciences. All rights reserved. Pesticide Resistance: Strategies and Tactics for Management. 1986, National Academy Press, Washington, D.C. FACTORS INFLUENCING THE EVOLUTION OF RESISTANCE GEORGE P. GEORGHIOU and CHARLES E. TAYLOR Any attempt to devise management strategies for delaying or fore-stalling the evolution of pesticide resistance requires a thorough understanding of the parameters influencing the selection process. The parameters known to influence this process in pest populations are presented systematically under three categories—genetic, biological/ecological, and operational—and their relative importance is discussed with reference to available case histories. INTRODUCTION More than 447 species of arthropods have now developed resistance to insecticides (Georghiou, this volume). The main weapon for countering this resistance has been the use of alternative chemicals with structures that are unaffected by cross-resistance. The gradual depletion of available chemicals as resistance to them developed has revealed the limitations of this practice and emphasized the need for maximizing the ”useful life” of new chemicals through their application under conditions that delay or prevent the development of resistance. To achieve this goal it is essential to understand the parameters influencing the selection process. It is well established that resistance does not evolve at the same rate for all organisms that come under selection pressure. Resistance may develop rapidly in one species, more slowly in another, and not at all in a third. For example, despite enormous selection pressure during many years of intensive DDT treatment in the corn belt of the United States, the corn borer showed no evidence of resistance. Yet house flies in many areas developed resistance POPULATION BIOLOGY OF PESTICIDE RESISTANCE: BRIDGING THE GAP BETWEEN THEORY AND PRACTICAL APPLICATIONS 157 Pesticide Resistance: Strategies and Tactics for Management Copyright National Academy of Sciences. All rights reserved. within two to three years under selection pressure by this insecticide. Even within a species, resistance may develop more rapidly in one population than in another. The Colorado potato beetle, for example, showed far greater propensity for resistance on Long Island than on the mainland (Forgash, 1981, 1984). TABLE 1 Known or Suggested Factors Influencing the Selection of Resistance to Insecticides in Field Populations A. Genetic a. Frequency of R alleles b. Number of R alleles c. Dominance of R alleles d. Penetrance, expressivity, interactions of R alleles e. Past selection by other chemicals f. Extent of integration of R genome with fitness factors B. Biological/Ecological 1. Biotic a. Generation turnover b. Offspring per generation c. Monogamy/polygamy, parthenogenesis 2. Behavioral/Ecological a. Isolation, mobility, migration b. Monophagy/polyphagy c. Fortuitous survival, refugia C. Operational 1. The chemical a. Chemical nature of pesticide b. Relationship to earlier-used chemicals c. Persistence of residues, formulation 2. The application a. Application threshold b. Selection threshold c. Life stage(s) selected d. Mode of application e. Space-limited selection f. Alternating selection SOURCE: Adapted from Georghiou and Taylor (1976). There are many factors that can influence the rate at which this evolution proceeds. One effort to systematize them is shown in Table 1, modified slightly from a classification we proposed and discussed earlier (Georghiou and Taylor, 1976, 1977a,b). The factors are grouped into three categories, depending on whether they concern the genetics of resistance, the biology/ ecology of the pest, or the control operations used. Most factors in the first two categories cannot be controlled, and the importance of some may not even be determined until resistance has already developed. Only through POPULATION BIOLOGY OF PESTICIDE RESISTANCE: BRIDGING THE GAP BETWEEN THEORY AND PRACTICAL APPLICATIONS 158 Pesticide Resistance: Strategies and Tactics for Management Copyright National Academy of Sciences. All rights reserved. hindsight, for example, can one obtain any idea about the initial frequency of the alleles conferring resistance. Nor is it usually possible to measure dominance until one isolates such alleles and makes the appropriate crosses. In some cases these issues may be addressed in laboratory studies where resistant strains can be developed by selection on large, recently colonized populations. Nonetheless, some factors that influence the evolution of resistance are under man’s control, especially those related to the timing and dose of insecticide application (Operational Factors, Table 1). The problem is to identify them and determine how their manipulation under the existing genetic and biological/ ecological constraints may retard the evolution of resistance. During the past few years, important contributions have been made by workers in a handful of laboratories, mainly in the United States, the United Kingdom, and Australia (Comins, 1977a,b, 1979a,b; Georghiou and Taylor, 1977a,b; Haile and Weidhaas, 1977; Curtis et al., 1978; Conway and Comins, 1979; Sutherst and Comins, 1979; Sutherst et al., 1979; Taylor and Georghiou, 1979, 1982; Gressel and Segel, 1982; Muggleton, 1982; Tabashnik and Croft, 1982; Levy et al., 1983; McPhee and Nestmann, 1983; Taylor et al., 1983; Wood and Cook, 1983; Knipling and Klassen, 1984; Mani and Wood, 1984; McKenzie and Whitten, 1984). Some of these contributions are examined in other papers in this symposium. We shall confine ourselves to a discussion of how, in a historical perspective, the accumulated knowledge on the occurrence and dynamics of resistance leads to the recognition of these factors (Table 1) as important. GENETIC FACTORS IN RESISTANCE Evolutionists frequently assume that organisms have the capacity to evolve nearly any type of resistance. From this follow many of the “optimization” arguments and the “adaptationist program” (Lewontin and Gould, 1979). This assumption is not warranted for insecticide resistance. Some populations obviously do not have the capacity to come up with the necessary resistant alleles in the first place, despite what would seem to be an obvious advantage for doing so. The corn borer is one species that did not. The paucity of cases of resistance to arsenicals in insects and to copper fungicides in plant pathogens are other examples. It has been speculated that herbivorous species, which have frequently evolved the capacity to deal with plant alkaloids, are in some sense preadapted to dealing with the problems posed by dangerous chemicals in their environment (Croft and Brown, 1975). Related to this is the fact that there may be many ways to achieve resistance —by detoxifying the chemicals, altering site specificity, reducing penetration, behavioral avoidance of residues, to name a few. When more avenues are open it would be expected that resistance would evolve more easily. Once alleles conferring resistance are present in the population, the fre POPULATION BIOLOGY OF PESTICIDE RESISTANCE: BRIDGING THE GAP BETWEEN THEORY AND PRACTICAL APPLICATIONS 159 Pesticide Resistance: Strategies and Tactics for Management Copyright National Academy of Sciences. All rights reserved. quency at which they occur may be important. There are several reasons for this. Obviously if the initial frequency is higher, then resistance has a head start. There may, however, be an Allee effect, so if the population is reduced to a sufficiently low level, the resulting population size is too small to sustain positive growth, perhaps by failure to find mates. More important, the selection pressures and immigration rates may impose an unstable equilibrium of gene frequencies, below which resistance alleles decrease in fitness and above which they increase (Haldane, 1930). In this case the initial frequency is especially important. In practice the importance of many factors for resistance seems related to this unstable equilibrium. In the simplest instance this equilibrium depends largely on initial gene frequency, dominance, and immigration. These factors in turn may depend on others. Imagine a population with resistant allele, R, at a low frequency. Homozygous RR individuals may occur if the population is large enough, but will be very few in number. If the resistance is recessive or can be made recessive by application of an appropriately high dose of insecticide (Taylor and Georghiou, 1979), then following insecticide use all of the susceptible homozygotes (SS) and heterozygotes (RS) will be eliminated, leaving only the very few RR. If now there is an inflow of largely susceptible migrants, then those few RR will mate with SS homozygote immigrants, and the offspring for the next generation will be almost all SS and RS. These can be killed with another application of insecticide, keeping the population under control. It is possible to study this result mathematically and describe precisely when it should be observed (Comins, 1977a; Curtis et al., 1978; Taylor and Georghiou, 1979). It is generally thought that resistance alleles are mildly deleterious prior to insecticide use, so that they are present initially at some sort of mutationselection balance. This would typically be at an allele frequency of 10-2 to 10-4, with the RR homozygotes present at 10-4 to 10-8. Of course if two loci are required or if more than one nucleotide change is necessary then the frequency may be substantially less (Whitten and McKenzie, 1982). McDonald (1959) proposed that dieldrin resistance, being more dominant than DDT resistance in Anopheline mosquitoes, would evolve at a faster rate. In theory there should be little difference between rates of evolution of dominant and recessive alleles in the absence of immigrants. But, in fact, McDonald’s prediction has been more-or-less realized. The reason for this is probably related to the unstable equilibrium described above, which exists only when the resistant allele is recessive. Dominance typically depends on the dose applied. Figure 1 shows the dosage-response curves for three genotypes of a mosquito, Culex quinquefasciatus , exposed to a pyrethroid insecticide. When a small dose, Ds, is applied, the heterozygotes survive, but with a larger dose, DL, they do not. Thus, with Ds, the resistance is functionally dominant, but with DL, it is POPULATION BIOLOGY OF PESTICIDE RESISTANCE: BRIDGING THE GAP BETWEEN THEORY AND PRACTICAL APPLICATIONS 160 Pesticide Resistance: Strategies and Tactics for Management Copyright National Academy of Sciences. All rights reserved. functionally recessive. Modifier genes are known to change the location of the heterozygote line, typically moving it to the right. Figure 1 Dosage-response lines for larvae of Culex quinquefasciatus susceptible, heterozygous, and resistant tested with permethrin. The dominance is seen to depend on dose: with a small dose (Ds), resistance is functionally dominant, whereas with a large dose (D L) it is functionally recessive. Modifier genes may be important in other ways as well, most notably by helping to integrate the resistance allele into the rest of the genome to produce a “harmoniously coadapted genome” in the sense of Mayr (1963) or Dobzhansky (1970). There may be many pleiotropic effects from the substitution of a resistant allele for its wild-type alternative. Many of these are likely to be detrimental, so the resistant allele is initially mildly deleterious (Ferrari and Georghiou, 1981). Later, when there has been an opportunity for the modifiers to be selected and the pleiotropic side effects have been compensated for, such a disadvantage diminishes or disappears. With few exceptions resistant populations demonstrate lower fitness than their susceptible counterparts. Continued selection may improve fitness through coadaptation of the resistant genome, resulting in more Stable resistance. A dramatic illustration of this is a laboratory experiment of Abedi and Brown (1960). They selected for resistance, then released selection, then selected, and so forth. After several cycles resistance evolved much more rapidly and was more stable than initially. Almost certainly, modifier genes were the cause. Instability of resistance may not necessarily be due entirely to differences in fitness, however. For example, genes for resistance to an organophosphate (temephos), a pyrethroid (permethrin), and a carbamate (propoxur) were introduced into a susceptible strain of Culex quinquefasciatus through a POPULATION BIOLOGY OF PESTICIDE RESISTANCE: BRIDGING THE GAP BETWEEN THEORY AND PRACTICAL APPLICATIONS 161 Delaying insect resistance to transgenic crops Bruce E. Tabashnik1 Department of Entomology, University of Arizona, Tucson, AZ 85721 I n her seminal work, Silent Spring, Rachel Carson writes: ‘‘If Darwin were alive today the insect world would delight and astound him with its impressive verification of his theories of survival of the fittest. Under the stress of intensive chemical spraying the weaker members of the insect populations are being weeded out.’’ (1) Evolution of insecticide resistance in 400 species of insects not only confirms Darwin’s theories, it threatens agriculture and human health worldwide (www. pesticideresistance.com/; ref. 2). To reduce reliance on insecticide sprays, corn and cotton have been genetically engineered to produce insecticidal crystal (Cry) proteins derived from the bacterium Bacillus thuringiensis (Bt). Transgenic Bt corn and Bt cotton grew on 42 million ha during 2007, with a cumulative total of 200 million ha planted worldwide since their commercialization in 1996 (3). However, the history of insecticide resistance informs us that adaptation by insects could diminish the long-term efficacy of Bt crops and the associated economic, health, and environmental benefits (4–6). To date, field-evolved resistance to Bt crops has been documented in only 3 insect species (Fig. 1) (7–10). Along with other evidence, the report by Meihls et al. (11) in this issue of PNAS suggests that refuges of plants that do not produce Bt toxins may be useful for delaying insect resistance to Bt crops. The refuge strategy, which is mandated in the United States and elsewhere, is based on the idea that most of the rare resistant pests surviving on Bt crops will mate with abundant susceptible pests from nearby refuges of host plants without Bt toxins (12, 13; www.epa.gov/EPAPEST/1998/January/Day-14/paper.pdf). If inheritance of resistance is recessive, the hybrid progeny from such matings will die on Bt crops, substantially slowing the evolution of resistance. This approach is sometimes called the ‘‘high-dose refuge strategy’’ because it works best if the dose of toxin ingested by insects on Bt plants is high enough to kill all or nearly all of the aforementioned hybrid progeny (12, 13). In principle, if a high dose is not achieved, resistance can be delayed by increasing refuge abundance, which lowers the proportion of the population selected for resistance to compensate for survival of hybrid progeny on Bt plants (12, 13). The most direct way to test the highdose hypothesis is to let resistant and susceptible adults mate in the laboratory and measure survival of their hybrid progeny on Bt plants. Because suitable resistant strains for direct tests usually are not available, indirect tests are used. One such method relies on the reasonable assumption that if Bt plants do not kill virtually 100% of susceptible individuals, they probably will not kill nearly all hybrid individuals. Thus, the U.S. Environmental Protection Agency guidelines for a high dose specify that Bt plants should kill at least 99.99% of susceptible insects in the field (www.epa.gov/scipoly/sap/meetings/ 1998/0298mtg.htm). Meihls et al. (11) studied a case in which the high-dose standard is not satisfied: resistance of western corn rootworm, Diabrotica vergifera vergifera, to transgenic corn producing Bt toxin Cry3Bb. This devastating beetle pest and closely related species cost U.S. farmers approximately $1 billion annually (14). Important advances incorporated by Meihls et al. (11) include use of Bt corn plants in the greenhouse to select rootworm colonies for resistance and estimation of larval survival in the field on Bt corn plants relative to nearly identical (‘‘isoline’’) non-Bt corn plants. The failure to achieve a high dose was indicated first by the survival of susceptible rootworm larvae on this type of Bt corn. Meihls et al. confirmed this conclusion by showing that survival in the greenhouse on Bt corn relative to non-Bt corn was 48–73% for hybrid progeny of resistant and susceptible adults. Supporting predictions from the refuge theory, Meihls et al. (11) report that resistance evolved quickly without refuges and slower or not at all with refuges. They exposed rootworm colonies to Bt corn in the greenhouse under 4 selection regimes: constant exposure, neonate exposure, late exposure, and no exposure (control). The constant-exposure colony was reared on Bt corn throughout the larval development period. Larvae in the neonateexposure colony were placed on Bt corn as neonates, then shifted to non-Bt corn to complete development. Larvae in Author contributions: B.E.T. wrote the paper. Conflict of interest statement: Although preparation of this article was not supported by organizations that may gain or lose financially through its publication, the author has received support for other research from Monsanto, Cotton Inc., the Cotton Foundation and the Arizona Cotton Growers Association. The author is a coauthor of a patent application filed with the World Intellectual Property Organization on engineering modified Bt toxins to counter pest resistance, which is related to published research [Science (2007) 318:1640 –1642]. See companion article on page 19177. 1E-mail: [email protected]. © 2008 by The National Academy of Sciences of the USA Fig. 1. Global adoption of Bt crops and evolution of insect resistance. The cumulative total planting of Bt crops worldwide was 200 million ha from 1996 to 2007 (3), but so far, resistance in the field has been detected in only 3 lepidopteran species: Helicoverpa zea (bollworm), to Bt cotton producing Cry1Ac in the southeastern United States in 2003 (7), Spodoptera frugiperda (fall armyworm) to Bt corn producing Cry1F in Puerto Rico in 2006 (9), and Busseola fusca (stem borer) to Bt corn producing Cry1Ab in South Africa in 2006 (8), depicted in the photo (Courtesy: Prof. Johnnie van den Berg, North-West University, Potchefstroom, South Africa). www.pnas.orgcgidoi10.1073pnas.0810763106 PNAS December 9, 2008 vol. 105 no. 49 19029 –19030 COMMENTARY Downloaded at UNIV OF CALIFORNIA RIVERSIDE on November 1, 2020 the late-exposure colony ate non-Bt corn as neonates and completed development on Bt corn. After 3 generations of selection, the constant exposure colony was highly resistant; its survival on Bt corn plants was similar to survival of the control colony on non-Bt corn plants. In marked contrast, after 3 generations of selection, the neonate- and late-exposure colonies remained as susceptible to Bt corn as the control colony. After 6 generations of selection, survival on Bt corn increased significantly in the late-exposure colony, but not in the neonate-exposure colony. Meihls et al. (11) suggest that the 2 partial-exposure regimes might emulate situations in the field where refuges are provided by non-Bt corn or grassy weed host plants interspersed with Bt corn. The results of Meihls et al. (11) parallel those of Lefko et al. (15) showing that in greenhouse experiments without refuges, rootworm rapidly evolved resistance to transgenic corn producing Bt toxins Cry34Ab and Cry35Ab, which also does not meet the high-dose standard (11). It remains to be seen what will happen in the field where refuges of at least 20% non-Bt corn are required (www.epa.gov/ opp00001/biopesticides/ingredients/ factsheets/factsheet006484.htm). Bt corn producing Cry3Bb for rootworm control was registered in 2003 and first exceeded 1 million ha planted in the United States in 2005 (www.monsanto.com/pdf/investors/ 2008/2008biotechacres.pdf). The more extensive field experience since 1996 with Bt corn and cotton producing Cry1 toxins for caterpillar control is helpful for putting the rootworm results in context. Analysis of more than a decade of resistance monitoring data for 6 major caterpillar pests targeted by Bt corn and cotton suggests that the principles of the refuge strategy may apply in the field (10). Resistance to Bt cotton producing Cry1Ac was detected after 7–8 years in some field populations of Helicoverpa zea in the southeastern United States, but fieldevolved resistance to Bt crops was not found in the 5 other pests examined: Helicoverpa armigera, Heliothis virescens, Ostrinia nubilalis, Pectinophora gossypiella, and Sesamia nonagriodes (10). As with rootworm and Bt corn, tests with H. zea showed that Bt cotton producing Cry1Ac does not meet the high-dose standard, based on both survival of susceptible larvae on the transgenic plants and dominant inheritance of resistance (10). In contrast, Cry1Ac-producing cotton and Cry1Abproducing corn meet the high-dose criterion for the 3 other pests from the continental United States (H. virescens, O. nubilalis, and P. gossypiella) that have remained susceptible to Bt crops for 10 years. This comparison suggests that the lack of a high dose for H. zea may have favored its faster evolution of resistance. Field experience with caterpillar pests also suggests that if the high-dose standard is not met, increasing the abundance of refuges relative to Bt crops can delay resistance. For H. zea, higher refuge abundance was associated with slower resistance evolution in North Carolina compared with Arkansas and Mississippi (10). Analogously, relatively high abundance of non-Bt host plant refuges in Australia and China may have helped to slow H. armigera resistance to Bt cotton producing Cry1Ac (10). Recently, field-evolved resistance to Bt corn has been reported for 2 additional caterpillar pests: Busseola fusca resistance to Cry1Ab-producing corn in South Africa (8) and Spodoptera frugiperda resistance to Cry1F-producing corn in Puerto Rico (9). Although published data are limited for these instances, failure to achieve the high-dose standard and to implement adequate refuges may have hastened resistance (8, 9). To maximize knowledge gained from these and other cases, it is important for scientists in academia, industry, and government to make publicly available the relevant information on the efficacy of transgenic crops and refuge abundance. The first decade of transgenic crops producing Bt toxins for insect control has been successful, despite the few examples of resistance noted above. The second and subsequent decades of insecticidal transgenic crops will entail an increasing diversity of toxins, greater use of plants producing 2 or more distinct toxins, and other, novel approaches for countering insect resistance (16–18). The new data reported by Meihls et al. (11) can be used to refine models for predicting evolution of resistance to Bt corn by western corn rootworm. This pernicious pest has but 1 generation per year, which might slow its resistance evolution relative to caterpillar pests with multiple generations. It will be intriguing to determine whether, as observed so far with caterpillars, the field outcomes with beetles confirm the concepts of the refuge strategy. If so, this would bolster management of insect resistance to transgenic crops as a compelling illustration of applying evolutionary principles to benefit humankind. ACKNOWLEDGMENTS. The work was supported by Department of Agriculture grants CSREES-NRI 2006-35302-17365 and 2008-35302-0390. Alex Yelich and Cara Gibson provided assistance with graphics. 1. CarsonR (1962)Silent Spring(HoughtonMifflin,NewYork). 2. Onstad DW (2008) Insect Resistance Management: Biology, Economics and Prediction (Academic, London). 3. James C (2007) Global Status of Commercialized Biotech/GM Crops: 2007. ISAAA Briefs No. 37 (Ithaca, NY, International Service for the Acquisition of Agribiotech Applications). 4. Tabashnik BE (1994) Evolution of resistance to Bacillus thuringiensis. Annu Rev Entomol 39:47–79. 5. Cattaneo M, et al. (2006) Farm-scale evaluation of transgenic cotton impacts on biodiversity, pesticide use, and yield. Proc Natl Acad Sci USA 103:7571–7576. 6. Romeis J, Shelton AM, Kennedy GG, eds (2008) Integration of Insect-Resistant Genetically Modified Crops within IPM Programs (Springer, New York). 7. Luttrell RG, et al. (2004) Resistance to Bt in Arkansas populations of cotton bollworm. Proceedings of the 2004 Beltwide Cotton Conferences, San Antonio, TX, January 5–9, 2004, ed Richter DA (National Cotton Council of America, Memphis, TN), pp 1373–1383. 8. Van Rensburg JBJ (2007) First report of field resistance by stem borer, Busseola fusca (Fuller) to Bt-transgenic maize S African J Plant Soil 24:147–151. 9. Matten SR, Head GP, Quemada, HD (2008) How governmental regulation can help or hinder the integration of Bt crops into IPM programs. Integration of Insect-Resistant Genetically Modified Crops within IPM Programs, eds Romeis J, Shelton AM, Kennedy GG (Springer, New York), pp 27–39. 10. Tabashnik BE, Gassmann AJ, Crowder DW, Carrie` re Y (2008) Insect resistance to Bt crops: Evidence versus theory. Nat Biotech 26:199 –202. 11. Meihls LN, et al. (2008) Increased survival of western corn rootworm on transgenic corn within three generations of on-plant greenhouse selection. Proc Natl Acad Sci USA 105:19177–19182. 12. Gould F (1998) Sustainability of transgenic insecticidal cultivars: Integrating pest genetics and ecology. Annu Rev Entomol 43:701–726. 13. Tabashnik BE, Gould F, Carrie` re Y (2004) Delaying evolution of insect resistance to transgenic crops by decreasing dominance and heritability. J Evol Biol 17:904 –912. 14. Rice ME (2003) Transgenic rootworm corn: Assessing potential agronomic, economic, and environmental benefits. Plant Health Progress, 10.1094/PHP-2004- 0301-01-RV. 15. Lefko SA, et al. (2008) Characterizing laboratory colonies of western corn rootworm (Coleoptera: Chrysomelidae) selected for survival on maize containing event DAS-59122–7. J Appl Entomol 132:189 –204. 16. Baum JA, et al. (2007) Control of coleopteran insect pests through RNA interference. Nat Biotech 25:1322–1326. 17. Sobero´ n M, et al. (2007) Engineering modified Bt toxins to counter insect resistance. Science 318:1640 – 1642. 18. Bravo A, Sobero´ n M (2008) How to cope with insect resistance to Bt toxins? Trends Biotech 26:573–579. Plants that do not produce Bt toxins may be useful for delaying insect resistance to Bt crops. 19030 www.pnas.orgcgidoi10.1073pnas.0810763106 Tabashnik Downloaded at UNIV OF CALIFORNIA RIVERSIDE on November 1, 2020 Pesticide Resistance: Strategies and Tactics for Management Copyright National Academy of Sciences. All rights reserved. TABLE 1 Fitness Components of Resistant (R) Compared with Susceptible (S) Strains Species Insecticide Fecundity (R/S) Developmental Time (S/R) References Field Selected Resistant Strains Musca domestica DDT — 0.99 (NS) March and Lewallen (1950) M. domestica1 DDT 1.07 0.71a Pimentel et al. (1951) M. domestica DDT 0.83 (NS) 0.99 (NS) Babers et al (1953) M. domestica DDT (probably KDR) — 1.05 Bogglid and Keiding (1958) Anopheles albimanus Dieldrin 1.02 m Gilotra (1965) Blatella germanica Chlordane 0.88a 1.03 Grayson (1954); Perkins and Grayson (1961) Anthonomus grandis Endrin 0.96 (NS) 1.01 Bielarski et al (1957) Tribolium castaneum2 Malathion 0.19a — Brower (1974) Laboratory Selected Resistant Strains M. domestica DDT 0.67a 0.88a Babers et al (1953) B. germanica DDT 0.67a 0.94a Grayson (1953); Perkins and Grayson (1961) T. castaneum DDT 0.36a 0.86a Bhatia and Pradhan (1968) Dermestes maculatus Lindane 0.12a 0.85a Shaw and Lloyd (1969) A. grandis Endrin 0.78a 0.98a Thomas and Brazzel (1961) a R strain statistically less fit than S strain (p <.05). 1 Selected for five generations in laboratory. 2 Selected for 10 generations in laboratory. SOURCE: See references column. POPULATION BIOLOGY OF PESTICIDE RESISTANCE: BRIDGING THE GAP BETWEEN THEORY AND PRACTICAL APPLICATIONS 261
Get professional assignment help cheaply
Are you busy and do not have time to handle your assignment? Are you scared that your paper will not make the grade? Do you have responsibilities that may hinder you from turning in your assignment on time? Are you tired and can barely handle your assignment? Are your grades inconsistent?
Whichever your reason may is, it is valid! You can get professional academic help from our service at affordable rates. We have a team of professional academic writers who can handle all your assignments.
Our essay writers are graduates with diplomas, bachelor, masters, Ph.D., and doctorate degrees in various subjects. The minimum requirement to be an essay writer with our essay writing service is to have a college diploma. When assigning your order, we match the paper subject with the area of specialization of the writer.
Why choose our academic writing service?
Plagiarism free papers
Timely delivery
Any deadline
Skilled, Experienced Native English Writers
Subject-relevant academic writer
Adherence to paper instructions
Ability to tackle bulk assignments
Reasonable prices
24/7 Customer Support
Get superb grades consistently
Get Professional Assignment Help Cheaply
Are you busy and do not have time to handle your assignment? Are you scared that your paper will not make the grade? Do you have responsibilities that may hinder you from turning in your assignment on time? Are you tired and can barely handle your assignment? Are your grades inconsistent?
Whichever your reason may is, it is valid! You can get professional academic help from our service at affordable rates. We have a team of professional academic writers who can handle all your assignments.
Our essay writers are graduates with diplomas, bachelor’s, masters, Ph.D., and doctorate degrees in various subjects. The minimum requirement to be an essay writer with our essay writing service is to have a college diploma. When assigning your order, we match the paper subject with the area of specialization of the writer.
Why Choose Our Academic Writing Service?
Plagiarism free papers
Timely delivery
Any deadline
Skilled, Experienced Native English Writers
Subject-relevant academic writer
Adherence to paper instructions
Ability to tackle bulk assignments
Reasonable prices
24/7 Customer Support
Get superb grades consistently
How It Works
1. Place an order
You fill all the paper instructions in the order form. Make sure you include all the helpful materials so that our academic writers can deliver the perfect paper. It will also help to eliminate unnecessary revisions.
2. Pay for the order
Proceed to pay for the paper so that it can be assigned to one of our expert academic writers. The paper subject is matched with the writer’s area of specialization.
3. Track the progress
You communicate with the writer and know about the progress of the paper. The client can ask the writer for drafts of the paper. The client can upload extra material and include additional instructions from the lecturer. Receive a paper.
4. Download the paper
The paper is sent to your email and uploaded to your personal account. You also get a plagiarism report attached to your paper.
PLACE THIS ORDER OR A SIMILAR ORDER WITH Essay fount TODAY AND GET AN AMAZING DISCOUNT
The post Essay on microbial resistance to antibiotics appeared first on Essay fount.
What Students Are Saying About Us
.......... Customer ID: 12*** | Rating: ⭐⭐⭐⭐⭐"Honestly, I was afraid to send my paper to you, but you proved you are a trustworthy service. My essay was done in less than a day, and I received a brilliant piece. I didn’t even believe it was my essay at first 🙂 Great job, thank you!"
.......... Customer ID: 11***| Rating: ⭐⭐⭐⭐⭐
"This company is the best there is. They saved me so many times, I cannot even keep count. Now I recommend it to all my friends, and none of them have complained about it. The writers here are excellent."
"Order a custom Paper on Similar Assignment at essayfount.com! No Plagiarism! Enjoy 20% Discount!"
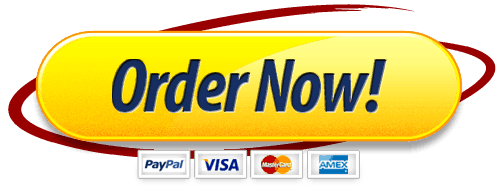